The quantum reason that ultra-massive stars are forbidden – Big Think
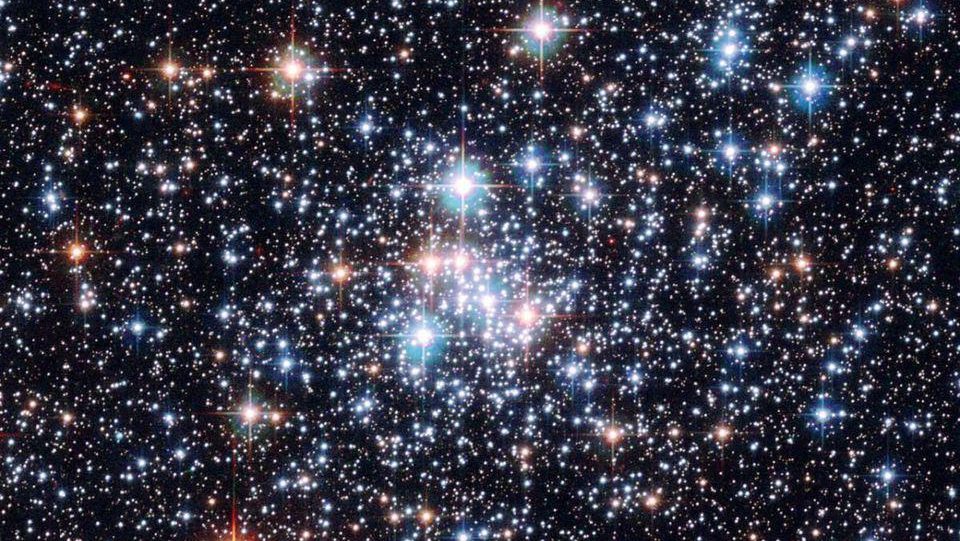
Here in our Universe, the most limiting factor behind the largest structures we see is time. Shortly after the Big Bang, there were no stars, galaxies, or black holes: objects that require significant amounts of mass to accumulate in one place. The Universe, even though it was born with density imperfections on all cosmic scales, only allows signals — even gravitational signals — to propagate through its space at a finite, limited speed: the speed of light. As a result, it takes a long period of time for enough mass to accumulate in order for these structures to emerge:Now that some 13.8 billion years have passed, we can see what the largest, most massive examples of these different types of objects are. Black holes range from about three solar masses up to tens of billions of solar masses. Galaxies can contain as little as a few hundred stars, but as many as many trillions. Galaxy clusters can be made up of thousands of large galaxies, containing quadrillions of solar masses. And cosmic filaments, such as various great walls, can contain up to 100,000 galaxies, approaching a quintillion solar masses at maximum.But stars? They come in with as little as 7.5% of the Sun’s mass, but the most massive star known is just 260 solar masses, and even in theory a star of thousands (or more) solar masses simply cannot be. The reason why, surprisingly, takes us deep into the heart of quantum physics.The problem with finding high-mass stars is twofold:The only individual stars we can observe directly are the ones that are close enough that we can distinguish them from other stars that are close to them; stars that are too distant will “blend together” with the light from other nearby ones, and as a result, we’ll only see the light from many stars, smeared together as we move from one pixel in an image to the adjacent one. This restricts us to looking at stars located within just a few million light-years for most ground-based telescopes, to stars within about 50 million light-years for the Hubble Space Telescope, and to stars within about 100-150 million light-years for JWST.The only exception to this rule comes from gravitational lensing: where foreground masses can bend, distort, and magnify the light from distant objects in the background. If you get very, very lucky, one of the “maximum magnification cusps” will happen to coincide with a very distant individual star. While we’ve gotten lucky in this way a couple of times with JWST, we didn’t get lucky enough to catch any stars that are more massive than the most massive ones we’ve found nearby.The second problem is also a challenge: the most massive stars that form are also the shortest-lived stars, and it’s important to understand why. Stars work by fusing light elements into heavier ones in their cores, through the process of nuclear fusion. This only occurs in the interior of stars if the temperatures are high enough: above a threshold of around 4,000,000 K. This explains the lower limit to stars: about 7.5% the mass of our Sun. Objects that are below that threshold — from about 1.5% to 7.5% of the Sun’s mass — still have core temperatures that rise over 1,000,000 K, which is enough to make them brown dwarfs (objects that undergo deuterium fusion), but not enough to make them stars by initiating standard hydrogen fusion.However, the more mass you accumulate on your star, the hotter the temperatures are that the star’s core achieves. While 4,000,000 K is enough to initiate nuclear fusion, the rate of fusion at that temperature is still very low. It’s why a star like Proxima Centauri, at around 12% of the Sun’s mass and very close to the low-mass end of stellar objects, emits just 0.16% of the Sun’s total energy. With a mass of 1.0 solar masses, our own Sun is more than 600 times as luminous as Proxima Centauri: emitting much more energy in any given period of time, as the rate of fusion inside our Sun is far greater than the rate of fusion within Proxima Centauri.Credit: LucasVB/Wikimedia Commons; Annotations: E. SiegelIn our Sun’s core, the temperatures achieved are much greater: around 15,000,000 K. At these temperatures, the rate of fusion is much, much greater (hundreds of times greater) than it is at a lower temperature of 4,000,000 K, and the trend continues: the greater your star’s core temperature, the faster its rate of fusion. As a result, more massive stars, which have higher core temperatures, are brighter and more luminous than lower-mass stars in general. Even though the Sun has about eight times as much mass as Proxima Centauri, its lifetime will be much shorter: whereas the Sun might live for 10-12 billion years, Proxima Centauri will live for somewhere around 5 trillion years, or about 500 times as long as the Sun.The same physics applies when we look to stars that are more massive than the Sun as well. Take a star like Sirius, the brightest star in the night sky. It’s only just over twice the mass of the Sun, but it’s more than 20 times as luminous. Sirius is also young: it’s less than 300 million years old, which is good. A star like Sirius will have a total lifetime that’s under 2 billion years, with a core temperature that’s even higher than that of our Sun. The more massive we go, the more luminous a star gets: powered by increased rates of nuclear fusion inside its larger, higher-temperature core. (Where the size of the core is determined by the size of the region inside the star where temperatures are 4,000,000 K or above.)This relationship gets more and more severe the more massive a star becomes. A star born with about 15 solar masses might only live for around 20 million years, while a star born with around 25 solar masses might live for under 10 million years before exhausting the hydrogen in its core. At the very high end, stars of over 100 solar masses might live for just 1 or 2 million years, including all of the evolved phases of its lifetime, before dying in a cataclysm such as a core-collapse supernova. Inside these very massive stars, core temperatures won’t stay in the millions or even the tens-of-millions of degrees, but will rise to hundreds-of-millions of degrees inside.The only location we can find these very massive stars is inside of regions where star-formation is still actively occurring. Stars form when giant molecular clouds of gas collapse, where the initially densest regions inside that cloud accrue more and more mass onto those clumps. The clumps that gravitationally grow the fastest attract more and more matter onto them, becoming the most massive stars that will form in that region. In the cosmic blink-of-an-eye, or just a few hundred thousand years, enormous numbers of new stars form with a wide variety of masses: hundreds, thousands, or even greater numbers of stars in very quick succession, depending largely on the initial mass of the molecular cloud in question.Here in the Milky Way, we’re largely familiar with the nearest large star-forming regions: the Orion Nebula, the Eagle Nebula, the Omega Nebula, and so on. But stars more than about 20 or 30 solar masses are very rare in these locations, as they don’t represent the most massive star-forming regions of all. Perhaps bizarrely, the largest star-forming region in the entire Local Group of galaxies isn’t located in the Milky Way or in Andromeda, but rather inside a much more modest galaxy: the Large Magellanic Cloud. Located around 168,000 light-years away, the Tarantula Nebula, also known as 30 Doradus, is nearly 2000 light-years across, and it marks the largest star-forming region — containing the most massive stars — ever discovered in our cosmic neighborhood.This star-forming region (above) is huge: with tens of thousands of new stars inside, and the largest star cluster presently known: NGC 2070, whose central concentration is further sub-named R136. Many of the most massive known stars are located in this concentration, with the most massive star of all, R136a1 (below), having a mass of around 200, and maybe up to 260, times the mass of our Sun. Despite being first identified way back in 1985, and then imaged with the Hubble Space Telescope in the 1990s, no star that’s more massive has ever been found. It shines at around five million times the brightness of our Sun, and the second brightest star in this cluster, R136a2, is located less than 0.1 light-years away from it.And yet, stars that achieve these high masses are exceedingly rare. You might think that something prevents more massive objects from forming; perhaps, you might reason, that once enough mass gathers and nuclear fusion ignites in a star’s core, it will emit winds and radiation that “blows away” any further matter that could fall onto it. It’s a nice idea, but it doesn’t work out in detail. Simulations indicate that streams of gas can form individual clumps of mass up that are up to tens of thousands of solar masses, and mergers of massive stars — especially in an ultra-dense star cluster like R136 — should be common, enabling the possibility of having stars of many hundreds of masses or even up to 1000 solar masses.So why don’t we have any?That’s where quantum physics comes in. Quantum physics doesn’t merely explain why the Sun shines, but also explains why stars “max out” at a certain mass. Remember, more massive for a star doesn’t just mean:but it also means greater rates-of-fusion in the star’s core and higher maximum temperatures inside the core. In particular, once a core temperature of about 300,000,000 K (or about 20 times the maximum core temperature inside the Sun) is reached, the majority of photon energy inside the star’s core is in the form of the most energetic types of photons of all: gamma rays.Credit: Dmitri Pogosyan/University of AlbertaWhen those gamma rays smash into other particles — protons, neutrons, heavier atomic nuclei, electrons, or other photons — there’s the opportunity to spontaneously create, if enough energy is available, an electron-positron pair: particles of matter and antimatter, respectively. The photons provide radiation pressure that holds the star up against gravitational collapse, and when you get to high enough temperatures that some of those photons start to spontaneously produce electron-positron pairs, that photon pressure drops.If the pressure only drops by a little bit, the star’s core will gravitationally contract by only a little bit, increasing the pressure and pushing the core back out. Not every star that spontaneously creates electron-positron pairs is in trouble; many of them can find (and oscillate around) an equilibrium state such as this.However, if the pressure drops by too much, then when the star’s core contracts, it can contract substantially, causing it to heat up to even higher temperatures. At higher temperatures, even more photons start producing electron-positron pairs, which causes the pressure to drop further. Now, with even less pressure, the core contracts further, heating up, causing more gamma rays to get absorbed, dropping the pressure further, etc. This continues until the star’s core heats up to a critical threshold: enabling a runaway fusion reaction, triggering a special type of supernova known as a pair-instability supernova.It’s thought that this is the fate for most stars over 130 solar masses, but not for the absolute most massive ones. Once you reach the mass of a star like R136a1 or greater, a new quantum process can occur in abundance: photodisintegration. If a high-enough energy gamma ray strikes a heavy atomic nucleus, it can cause that nucleus to enter an excited state. Instead of simply de-exciting (and emitting a gamma ray of the same energy), that excited state can decay by knocking out one (or more):out of the original nucleus.Imagine a similar scenario to the one we considered before: where a very massive star, with very high core temperatures, begins to produce these very high-energy gamma rays in its core. Some of those gamma rays will produce electron-positron pairs, reducing the core’s pressure, but now additionally, some of those gamma rays are absorbed by atomic nuclei and instead kick out nuclear particles.Guess what?Those gamma rays are now gone, and that causes the radiation pressure to drop in a one-way event; unlike electron-positron production, where every positron will re-annihilate with an electron to produce (pressure-creating) gamma rays again, that pressure is just now lower. As a result, no supernova ensues, and we simply get a direct collapse event, where the star’s core, or even the entire star itself, winks out of existence to become a black hole.And that’s it! That’s the limiting set of factors right there. Inside all stars, it’s radiation pressure that holds up the star in general — and the core in particular — against gravitational collapse. As the mass of your star increases, so does its core temperature, which shifts a greater percentage of those radiation pressure-providing photons into the gamma ray portion of the spectrum. Once that core temperature exceeds a few hundred million degrees, enough gamma ray photons begin converting into electron-positron pairs so that the radiation pressure begins to drop, and at still higher temperatures, those photons get absorbed by atomic nuclei, kicking out lighter nuclear particles and lowering the pressure even further.This means that all stars above a critical core temperature are fundamentally unstable, with the only questions being:In either case, it’s quantum physics, where high energy gamma rays spawn electron-positron pairs or where they collide with heavy atomic nuclei and spit out lighter nuclear particles, that causes the core pressure to drop and leads to an ensuing cataclysm. Above a critical mass threshold, there simply aren’t any stars that can overcome these limitations that are fundamental to physics.
Source: https://bigthink.com/starts-with-a-bang/quantum-reason-ultra-massive-stars-forbidden/