Ask Ethan: Could dark matter be “normal stuff” we can’t see? – Big Think
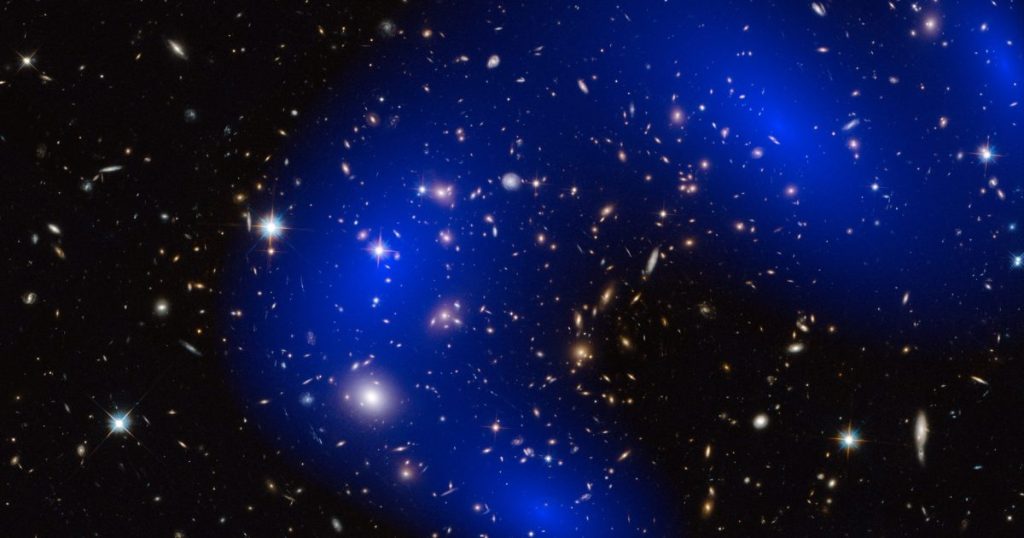
Out there in the Universe, our cosmic inventory is divided up into two categories:While normal matter makes up all the things we’ve ever directly detected and interacted with, including atoms, gas, dust, plasma, stars, planets, black holes, neutrinos, light, and even gravitational waves, that only sums up, in total, to about 5% of the total energy of the Universe. The overwhelming majority of the cosmic energy budget comes to us in the form of dark stuff, with dark energy (68%) dominating dark matter (27%) for the past few billion years.Even though dark energy and dark matter dominate most of the Universe, we’ve only ever detected them indirectly, leading many to doubt, or at least question, their existence. That’s what Richard Whitrock is asking about this week as well, writing in to inquire:“[is there] a measurable explanation based on aggregation; basically, that areas of space that we are not able to measure any electromagnetic radiation or gravity from actually have some, but it’s from incredibly small particles that are too far away to be measurable but can aggregate together. Essentially, dust or even subatomic particles that are too far spaced and too small for us to be able to see or measure from our location with our current tech, but is not a fundamentally exotic type of matter/energy.”So could that be it: could undetected radiation or matter, perhaps in places we haven’t yet been able to look, explain the “missing matter” or “missing component to the Universe’s expansion” that we know must be there? Let’s find out.When you look at any object in the Universe, there are two main ways we can think of to measure how much “stuff” is there, total, inside of it. The first is that we can look at the electromagnetic (i.e., light) signatures that come from the object itself. If there are stars, active black holes, shocked and heated gas, or any other sources of matter that can emit light, we can detect that light, figure out its origin, and infer how much mass there is in the form of the objects that emit that light. Additionally, we can look at the emitted light from any source that passes through a region with normal matter within it, and look for signatures like excited atoms or ground-state atoms that absorb at specific wavelengths of light, and infer their presence and abundance from those signatures, too. But there’s a second way to probe the Universe: with gravity, rather than with light. Sure, we can only absorb and detect photons, or other forms of light, directly (with the rare exceptions of cosmic particles or gravitational waves emitted from specific classes of events), but when that light is either created inside a region with a specific amount of gravity or travels through a region where the gravitational potential changes with time, those are effects we can directly detect. These gravitational effects, including gravitational redshift, gravitational blueshift, the motion of objects in a gravitational field, and gravitational lensing, give us probes of the Universe that are insensitive to what type, or species of matter is present, but rather only care about the total mass that’s there.It might be tempting, then, to look at the sources of light that are out there on cosmic scales and note that they’re primarily clumped and clustered together in stars and galaxies, and that those galaxies are further clumped and clustered together into galactic groups, galaxy clusters, and large-scale cosmic filaments. You might reason that, sure, these are locations where there’s lots of normal matter, but also lots of empty space between those collections, or clumps, of normal matter. And therefore, you might think, if normal matter makes up just ~5% of the Universe and dark matter makes up ~27%, that maybe that’s where the dark matter is hiding: in the form of normal matter that doesn’t emit or absorb light, but that’s still lurking there, somewhere, beyond the limits of our direct detection.It’s certainly a possibility that we can imagine, at least, to an extent. After all, the first hint we ever got of dark matter came way back in the 1930s, when astronomer Fritz Zwicky was studying the individual galaxies present within the Coma Cluster: the largest, densest, most massive cluster of galaxies known to exist within about 400 million light-years of Earth. Zwicky began examining the individual galaxies within that cluster, and measured their redshift: how much the light from them was shifted, owing to both the cosmic expansion of the Universe as well as their individual motions relative to one another.On average, he found that all of the galaxies within the cluster were receding from us at a fairly large speed: somewhere around 7000 km/s, when you aggregate them together. But individually, he found that there was a large distribution for the implied motion of any one galaxy in particular. Most of them moved at somewhere close to 7000 km/s: several hundred km/s either above or below that mark, but there were others, and a substantial number of them that moved away from us at only 6000 km/s or less, and an equal number that moved away from us at 8000 km/s or more. It was like a large number of galaxies were being pulled toward us, by some extra amount over the average, and an equally large number were being pushed away by an equivalent amount.This was an example, Zwicky realized, of that “second class” of measurements: a type of measurement that could allow you to infer the total mass of the cluster, as there’s a certain amount of mass that the cluster must possess in order for those fast-moving galaxies to remain gravitationally bound to it. The faster these speeds are for the individual galaxies within the cluster, even at the extremes, the more massive it implies the cluster’s total mass must be. For the Coma Cluster, in particular, there ought to be at least several hundred trillions worth of solar masses inside, all totaled together, approaching a whopping quadrillion solar masses overall.Where is that mass located?Is it:From Zwicky’s original method, alone, we wouldn’t be able to tell. Fortunately, we have another modern tool at our disposal that’s far more precise and comprehensive: gravitational lensing.Gravitational lensing isn’t sensitive to the type of mass that’s present: whether it’s normal matter, neutrinos, dark matter, or any other type of massive substance. What it is sensitive to is two things: how much mass there is within any particular bound structure, and how that mass is distributed along our line-of-sight. We can perform this type of lensing reconstruction for all sorts of galaxy clusters, using a combination of strong gravitational lensing, which creates arcs, rings, and multiple images of objects, as well as weak gravitational lensing, which distorts the shapes and ellipticities of background galaxies. When we perform this type of analysis for galaxy clusters, including the Coma Cluster, we find that the mass is primarily distributed in a diffuse, large halo that includes, but also goes beyond, the visible extent of galaxies in the cluster itself. Because we know how to look for absorption and emission signatures of matter, including from ionized matter such as plasmas, we can rule out the notion that this is normal matter of any type. If it were normal matter that were diffuse enough to fall below our detection threshold, there wouldn’t be enough of it to cause the lensing signal that we see.Furthermore, the fact that the mass remains in a diffuse, “fluffy” halo even this late in cosmic history, more than 13 billion years after the Big Bang, we can infer that this matter cannot be made of “normal” stuff like protons, neutrons, and electrons; it has to be something that neither absorbs, emits, nor collides with itself or with atom-based particles. It doesn’t necessarily have to be something beyond the Standard Model — for instance, it could be neutrinos — based on this type of observation alone, but it does rule out the “maybe it’s normal stuff that’s just non-luminous” explanation. 80% or more of the Coma Cluster must be in the form of dark matter, and this same relationship holds similarly true for other clusters.But on even larger cosmic scales, the case against dark matter, and attempts to explain our observations with “normal matter that’s just dark” fails colossally, and even worse than that, it fails on the scales of galaxy clusters. For one, there’s the large-scale cosmic web, which determines how matter clusters, overall, within our Universe. You would get a very, very different Universe in terms of the structures that formed within it if normal matter were all we had.That’s because there’s a feature known as baryon acoustic oscillations that shows up in the cosmic web: the oscillations that arise because normal matter — made of protons, neutrons, and electrons — interacts with, collides with, exchanges momentum with, and feels the pressure from photons, or particles of radiation. When you have a clump of matter, particularly at early times in cosmic history, that’s attempting to collapse under its own gravity, it’s that radiation pressure from photons that pushes back against it.On the other hand, if there’s a species of matter (i.e., dark matter) that doesn’t have those interactions, then not only won’t it gravitationally collapse, but it won’t make those oscillations. Instead, it will work to wash those oscillations out, minimizing their effects. When we observe the large-scale structure that’s arisen in the Universe today, on all cosmic scales, we see overwhelming evidence for the effects of dark matter by seeing how small those oscillations are and how severely damped they are.We can also look to the cosmic microwave background, which is a severe, stringent test of models of the Universe that don’t possess dark matter. Those “acoustic oscillations” we mentioned earlier show up for normal matter in a specific, predictable way. However, if there’s a type of mass that doesn’t have those interactions (i.e., dark matter), then it will simply gravitate and coast, failing to collide with, interact with, or exchange energy and momentum with anything else: normal matter, light, neutrinos, even other dark matter particles. This means, when we look at the temperature imperfections in the cosmic microwave background, we can search for correlations between temperature departures from the average on a variety of cosmic scales.If there were only normal matter and no dark matter, we’d get a specific set of features: peaks and valleys of a particular set of magnitudes and ratios that showed up in these temperature fluctuations. If there were both normal matter and dark matter, however, we’d get a very different set of features; a set of features whose number of oscillations and the relative strengths of their peaks and valleys depended on the absolute amount of normal matter and dark matter each, as well as their relative ratio. When we examine those features — using data that’s been available for nearly 25 years, since the first data release of WMAP — they again overwhelmingly show us that a Universe without dark matter is disallowed.But there’s an even more important test: what’s known as Big Bang Nucleosynthesis. Early on in cosmic history, the Universe was so hot and dense that it was an ionized plasma, with the earliest (and hottest, and densest) times corresponding to an era where no complex atomic nuclei could form: just bare protons and neutrons.If all we had were protons and neutrons, they would fuse together to build up heavier atomic nuclei: the cores of the elements of the periodic table. But because there are also photons present — the quanta of light — and they’re also ultra-energetic, they can blast any complex nuclei that form apart.Sure, at some point, the Universe will have expanded and cooled enough so that nuclear fusion can proceed, and it will. The ratios and species of the elements and isotopes we wind up with will then depend only on:That’s it. Because we understand the theory behind how normal matter interacts so well, and we’ve measured the light elements and their abundances so well, this explicitly allows us to measure the total abundance of normal (baryonic) matter in the Universe, as well as the abundance of radiation-in-the-form-of-photons in the Universe as well. (At least, from the Big Bang, as opposed to from stars.)All of this, together, tells us two very important facts about the Universe. It tells us how much normal matter there is, total, and that comes out to precisely 4.9% of the critical density of the Universe, with an uncertainty of just ±0.1%. And it tells us how much radiation there is in the form of photons (at least, prior to the formation of stars), and that number is known very precisely as well: about 0.08% of the critical density.Based on those facts, it renders it a complete impossibility, from an energy perspective, for dark matter or dark energy which comprise 95% of the cosmic energy density to be composed of the normal stuff, as it would have shown up in these other measurements. Yes, there are other reasons we know dark matter and dark energy exist, and other lines of evidence ruling out “normal” contributions to their effects, but:are four independent lines of evidence that all not only rule out a conventional explanation for dark matter and dark energy, but quantify precisely how much “normal stuff” there is in the Universe. No, dark matter and dark energy can’t just be “normal stuff” that’s dark, and these observations are how we can robustly rule that scenario out.Send in your Ask Ethan questions to startswithabang at gmail dot com!
Source: https://bigthink.com/starts-with-a-bang/dark-matter-normal-stuff-cant-see/